Part electricity and part chemistry, the brain's language relies on precision in both wiring and timing. Technologies such as EEG and fMRI let researchers and clinicians eavesdrop on the brain's internal conversations, but they miss many of their subtleties. And because scientists can't always parse the brain's code, the source of the communication breakdown in disorders like autism, depression and Parkinson's disease continues to elude them.
Compare that to, say, the heart, says Karl Deisseroth, in which the model of how the organ works—and what happens when it malfunctions—is relatively straightforward. "[With] congestive heart failure, the heart is not pumping well," he says. "We don't have that analogous understanding of the brain. What's really not working well in a disease state?"
As an associate professor of bioengineering and psychiatry at Stanford, Deisseroth bridges what some might call the study of the mind and the study of the brain. His work with clinical patients shows him the subjective, experiential side of the mind's maladies, but he is best known for his role in developing optogenetics, a new technology that gives scientists an extraordinarily precise tool for probing those disorders' biological origins.
In the murky world of neuroscience, optogenetics is literally a bright point. Using different wavelengths of light, delivered via fiber optic cable, scientists can control the activity of the cells that send and receive information in the brain. Blue light activates targeted neurons; yellow light quiets them. Think of it as a universal remote that operates the body's tiniest circuits.
Even better, because optogenetics can be used in living, behaving lab animals—rodents and primates so far—scientists can observe the effects of toggling brain circuits on disease symptoms. Turn these neurons off, and what goes wrong? What happens when you crank them back up? Establishing causal links between brain circuitry and symptoms could lead to highly targeted therapies—more effective drugs, perhaps, or a new class of less invasive brain implants to treat disorders like Parkinson's disease.
At Stanford, Deisseroth's lab has used optogenetics to investigate the roots of schizophrenia, and along with Stanford School of Medicine neurosurgeon Jaimie Henderson, the problems underlying Parkinson's disease. The work of colleague Krishna Shenoy, an associate professor of electrical engineering and bioengineering, could help those with paralysis and traumatic head injuries.
Yet as tantalizing as such potential applications are, Deisseroth's ultimate vision is focused less on cures, and more on gaining fundamental insight into the brain. Optogenetics may finally give scientists a way not only to better interpret its rapid-fire language, but also to start talking back to it using the speed and precision of light.
The idea of using light to control the mammalian brain didn't originate with Deisseroth's lab; it had been considered, but not achieved, by many others. He keeps a copy of a 1979 issue of Scientific American on his desk, in which Francis Crick—of Watson and Crick, the team that first described DNA's double-helix—imagined such a precise tool. "He didn't say 'light,' but he said what we need is a way to control neurons specifically as they are embedded in intact tissue," says Deisseroth. "He later speculated that light or other things might be one way of doing it, but he had absolutely no idea of how that might be done."
For decades, a few researchers outside neuroscience had experimented with genes derived from algae and other microorganisms. Algae contain light-sensitive proteins called opsins that act as tiny gatekeepers, regulating the flow of charged ions across cell membranes. For example, Channelrhodopsin-2, which responds to blue light, allows positive ions in, activating the cell. Another protein, Halorhodopsin, which comes from a bacteria-like single-celled organism, allows negative ions to enter in response to yellow light, silencing the cell.
But how to get these opsins into neurons? The genes for these proteins can be packaged inside a virus and delivered to an animal's brain by injection, making nearby neurons sensitive to light. Attaching snippets of DNA called promoter tags ensures that the genes only become activated within desired kinds of cells, so that those cells alone respond to light from the fiber optic cable.
Early attempts, including unpublished efforts by Roger Tsien's biology lab at UC-San Diego, didn't work—they'd chosen the wrong algae genes. When Deisseroth's lab took up the challenge in 2003, some considered it a waste of time. He was turned down for a foundational grant because funders doubted he could achieve convincing results. Even with the right genes, making algae opsins operate in animal tissue was a long shot. The opsins, Deisseroth explains, "come from these microbes that are not brains, or anything like brains. They don't have the same machinery, they don't operate on the same speed, they need chemical cofactors to get them to work." Using them posed two problems—finding those necessary chemical catalysts, and the possibility that the animal's cells would reject these foreign proteins.
Yet right away, Deisseroth says, "Several things happened that we had no right to expect. One is that we didn't have to add any chemicals to get it to work." Additionally, the neurons tolerated the opsins well.
Within two years Deisseroth's lab had published its breakthrough 2005 paper. Demonstrations of optogenetics' power were astounding. One widely circulated video shows a lab mouse with a fiber optic cable inserted into the part of its motor cortex that controls left-side movement; a thin wire protrudes from its skull. When the cable glows blue, the mouse circles left. When the light is turned off, it stops. Optogenetics had produced, in essence, a remote-controlled mouse.
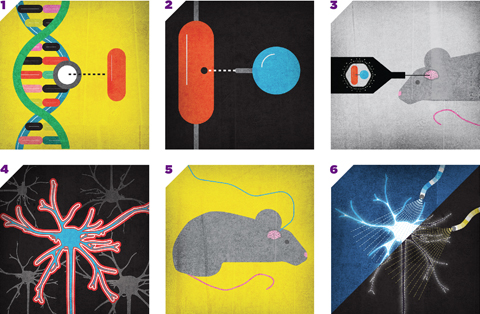
Illustrations by John Hersey
OPTOGENETICS 101
1. The gene that makes cells light-sensitive is isolated from algae or other microorganisms.
2. A snippet of DNA is added to "address" the gene to a particular type of neuron.
3. The tagged gene is inserted into a harmless virus and injected into the mouse's brain.
4. Targeted neurons express the light-sensitive protein on their surface.
5. Light is delivered via a fiber-optic cable wired into the mouse's brain.
6. Different proteins respond to particular wavelengths of light, turning neurons on or off.
The ability to switch cells off and on lends itself to an extraordinary number of potential applications, and Deisseroth's lab readily shared its technology. One group at Stanford is using it to explore the brain's sleep-wake circuits to gain insight into sleep-related disorders. One at UC-San Francisco is investigating the neural circuitry involved in addiction. Because optogenetics works on any cell, not just those of the brain, it also has been applied to organs such as the heart and the eye. Deisseroth has collaborated with European researchers who hope to restore vision to those with retinitis pigmentosa by using light-sensitive opsins to reactivate the eye's photoreceptors.
Perhaps the best-known optogenetics experiments are those exploring Parkinson's disease, possibly because they so clearly illustrate the contrast between the precision of optogenetics and older, more empirical, approaches. Doctors already have a highly successful implant technology for Parkinson's called deep brain stimulation (DBS) that ameliorates symptoms like tremor and rigidity. In this procedure, a metal electrode, sometimes referred to as a "pacemaker for the brain," is implanted into the subthalamic nucleus and pulses the area with electric current. (It's also in human clinical trials as a treatment for epilepsy and depression.)
In Parkinson's patients, the results can be dramatic. "It reduces symptoms by anywhere from 50 to 80 percent and reduces patients' medication needs by about 60 percent," says Stanford neurosurgeon Jaimie Henderson, who has performed approximately 600 such operations.
Improvement can be immediate. In a documentary video recording Henderson's operation on bike racer Davis Phinney, the cyclist's left arm shakes violently throughout the operation, but relaxes within seconds of the current being turned on. "That feels good," Phinney murmurs happily. "It's a relief, baby." Steven Gulie, who wrote about his own surgery under Henderson's care for Wired, described the flipping of the switch this way: "For more than five years my right hand hasn't felt the way it should. Suddenly, it's back. I can tap my fingers, move freely."
Yet DBS has drawbacks. Deisseroth has described electrodes as "fast but dumb." In the brain, different types of neurons are intermixed, and electricity floods them all. Neurons with the largest axons—the connective wiring that Henderson calls the "cabling of the brain"—absorb the most current, and electricity can take unexpected paths. That means side effects. "You can get tingling, muscle contractions, problems with speech, balance difficulties or cognitive problems," Henderson says.
What's more, nobody knows exactly why DBS works. It's not even clear which cells it affects. "The electrode could be driving excitatory cells; it could be driving inhibitory cells," Deisseroth says. "When you drive it this hard you can exhaust cells, so you might actually be inhibiting excitatory cells or you might be inhibiting inhibitory cells. Or you might be having some more complex effect like affecting synchrony or timing or the competition of two circuits for a third. With an electrode you just didn't know."
Most of all, it's not a cure. Even with treatment, patients deteriorate. DBS treats symptoms, but not their cause.
Optogenetics is helping doctors search for the underlying problem. In collaboration with Henderson, Deisseroth's lab used optogenetics to control Parkinson's symptoms in lab mice outfitted with fiber-optic implants. The researchers delicately toggled different neurons, and were surprised to find that the most therapeutic effect came not from stimulating a particular kind of cell, but from affecting the activity of their connective axons. When the axons were pulsed with light at high frequency, the mice behaved normally; when the pulse stopped, their symptoms returned. "This points to the concept that neuropsychiatric diseases may in large part relate to altered flow of activity between brain regions," says Deisseroth.
In other words, the problem isn't within the cells, it's in the informational flow among them. "One theory we are working with currently is that the brain communicates using different frequencies of oscillation," says Henderson. "Think of how radio works: There is a 'carrier' wave that is modulated by the sound that is to be carried, producing a complex waveform with lots of information. We think that the brain may get locked into rhythmical activity that blocks out that information and replaces it with a kind of 'hum,' as if fine-grained information in the radio waves is getting overwhelmed by a periodic repeating waveform. Once a circuit gets locked into that oscillation, you get abnormal behavior like seizures in epilepsy, and tremor and rigidity in Parkinson's."
Thanks in part to optogenetics, an emerging model of the brain holds that spatial specificity and millisecond timing are critical to information flow. If that's the case, then arrhythmia in the brain may be just as dangerous as it is in the heart. Deisseroth suspects that disruptions in timing and rhythm may underlie several brain disorders, including schizophrenia.
In one experiment, his group made a type of neuron called a fast-spiking cell light-sensitive. Rare in healthy brains, these neurons are even sparser in schizophrenics. But their function—and the ramifications of their deficiency—was not well understood. Using optogenetic controls, Deisseroth says, "We found that they play a role in a particular kind of brain wave called a gamma oscillation, which is a 40-times-per-second rhythm. They help maintain this rhythm, this synchronicity in the brain."
The group also demonstrated that this rhythmicity enhances communication in the brain; when the rhythm is off, messages are garbled. That's significant for schizophrenics, who have trouble processing sources of information and intent, often misreading the significance of objects and actions. Auditory hallucinations, or "voices in the head," also may be a result of misprocessed information, Deisseroth notes. "It may be a poorly recognized version of internal thoughts. Somehow the information that a thought is really coming from oneself is lost. It's viewed as a foreign thing, a voice speaking." Prior to the advent of optogenetics, he says, "There was no way to know this because there was no way to selectively control [the cells] on the right timescale."
The technique also may help restore communication in damaged brains. Stanford scientist Krishna Shenoy has spent the past 12 years working on brain-machine interfaces that enable paralyzed people to move prosthetic devices. These interfaces "read out" the brain's movement instructions, bypass the damaged spinal cord and send them directly to, for example, a robotic arm.
Interpreting the brain's signals is one thing, but "writing in" information about touch and the limb's location relative to the body is much harder. Imagine using a robotic arm to take a drink, he says: "When you move that arm out, all of a sudden you are immediately faced with the fact that you can't feel anything. If you squeeze on that glass too hard, you can shatter it. If you have a plastic water bottle, you may spill it. If you don't grip it hard enough, it may slip and fall."
Shenoy shares a $14.9 million DARPA grant to use optogenetics to develop a fundamental model of how the brain operates, and apply that insight to better understand how to write in information to the brain. While the grant's focus is on basic research, it could lead to very practical applications, such as more sensitive, naturally moving prosthetics, or perhaps treatments for traumatic head injuries. Learning how to write in the information that gets lost when a part of the brain is damaged could allow doctors to reroute signals around a damaged area.
In experiments with primates, Shenoy's lab is using tiny devices implanted atop the monkey's head that shine light down optical fibers implanted into the brain's surface. The light activates or silences neural circuits in the pre-motor and motor cortex, effectively giving instructions to the parts of the brain that initiate and control movement. "How are you going to inject information so precisely at the cellular level, talking to every type of cell in natural language?" Shenoy asks. "Optogenetics. That's certainly the newest, the most exquisite tool we have available as neuroscientists."
Deisseroth's office, tucked into the Clark Center basement, is nearly empty. It was recently renovated, and he's put off unpacking because he's enjoying the minimalism of three walls of bare shelving. The only objects on display are a trophy called "The Golden Brain" shaped like, well, a brain, and four empty champagne bottles, one to celebrate each major paper his team published this year. Stacks of boxes crowd around the door—some hold additional champagne bottles from previous years. The team has been extraordinarily prolific.
Deisseroth's career has been one of rapid successes: He went to college (biochemistry at Harvard) at age 16, medical school (at Stanford) at 20, and published his key optogenetics paper just a year after establishing his own lab. "Starting things a little too early has been a common theme my whole life," he says. He has a humble, almost boyish demeanor. As he speaks, he balls his fists up inside the hem of his faded T-shirt, and stares thoughtfully down at the table.
He's conscious of inhabiting an unusual middle ground between the abstraction of psychiatry and the precision of bioengineering. "They're worlds apart," he says. "Your average bioengineer has essentially nothing to say to your average psychiatrist and vice versa. But this is changing, and I think we're helping a little bit with that, because there is a natural affinity there."
Psychiatry, he says, can benefit from a bioengineering approach: "We need new tools, we need new technology, we need a quantitative understanding of the dynamics of these tiny electrical devices which are neurons as they operate within an enormously complicated circuit."
Deisseroth still spends about 20 percent of his time seeing clinical patients, including those with autism and depression. This, he says, "keeps me grounded in clinical reality" and helps him "maintain intuition for disease processes." He's hopeful that optogenetics could lead to new treatments—more accurately targeted drugs, better brain implants, perhaps more helpful talk therapy.
Functional brain imaging has shown physical differences in the brains of people who have, or have not, gone through therapy. "We may find that talk therapy can indeed, if it's done right, turn up or down particular brain circuits," he says. "So we might be able to even gain insight into what might be the best kind of talk therapy using optogenetic insight." He hopes that mental disorders will become less stigmatized as their origins are better understood.
Deisseroth is acutely sensitive to the moral implications of giving scientists such fine-tuned control over brain function. "The specificity of optogenetics raises the question of how precisely could one tweak a brain to really create an individual with different needs, desires, priorities, feelings," he says. And while he sees the promise of using the technique to, for example, help the severely depressed feel enjoyment again, he acknowledges that "there is a disturbing aspect, too, which raises questions of free will." (Recall the mouse that feels compelled to turn left, but doesn't know why.)
Even though optogenetic applications for humans are still entirely hypothetical, for bioethicists, the possibility of tinkering so intimately with the brain raises profound questions. "We're very brain-centric in our culture," observes Dr. Debra Mathews, the assistant director for science programs at the Johns Hopkins Berman Institute of Bioethics. The idea of intervening directly with what we see as the seat of our identities, and being able to influence personality and how we perceive ourselves, our ties to others and our roles in the world, is a sensitive one, she says, particularly given the sometimes inglorious history of psychosurgery.
Although optogenetics' selling point is extreme precision, Mathews cautions that any attempt to influence the brain is inherently unpredictable. "The brain is an incredibly complex organ, vastly more complex than anything else we deal with, and we can't guarantee that plucking at one cell is only going to affect the cells that we think it will, or the system we think we will, or the phenotypes—the characteristics—we think it will. We're just not that good yet."
Still, Mathews calls the possibility of using optogenetics to better understand the brain and look for new therapeutic targets "awesome." For her, the most exciting part of Deisseroth's work is its utility as a research tool. The technique "has the potential to be a pretty powerful tool for basic science, certainly for interrogating previously inaccessible questions, systems and connections in the brain."
Deisseroth agrees: "Before treatments, we need understanding."
Advancing understanding means enabling others to use optogenetics, a multidisciplinary skill set that requires expertise in fiber optics, virology, and animal surgery and behavior, among other disciplines. Deisseroth's group started training researchers in 2006 and has shared the technology with more than 700 labs worldwide.
This summer, with support from Stanford's BioX program, the University launched its Optogenetics Innovation Laboratory, offering free three-day or three-week training sessions to scholars from other universities. Deisseroth compares it to a microlending approach: "People can go back to their own institutions and teach other people." The goal is to train at least 100 researchers per year.
Freely sharing skills will lead to "geometric expansion" of the field, says neurosurgeon Henderson. "Rapid dissemination means that this incredibly cool technology can now be in the hands of hundreds of researchers" who can use it to test their own ideas. It's a way of leveraging the brainpower of the entire scientific community, Deisseroth notes: "I can't do even a tiny fraction of what I would want to do, and so the more people we can help the better."
Of course, there are potential roadblocks ahead. What works in mice and monkeys may not work in humans. There are also open questions regarding immune reactions, how deeply light spreads through brain tissue, and how well opsins will work over time. Optogenetics-derived therapies are far off, and will have to not only face the hurdle of FDA approval, but also prove superior to current treatments like deep brain stimulation.
But for Deisseroth, the ultimate goal is not using opsins in humans—it's gaining a fundamental understanding of the brain's complexities. Sitting in his near-bare office, with only a few commemorative champagne bottles to signify the accomplishments of the past few years, Deisseroth is proud of how far the field already has come in deciphering the brain's secret language. "If I had to shut down my lab tomorrow," he says, "I'd be a happy man."
KARA PLATONI is a frequent contributor who lives in Oakland.