At home, as his two young boys cycled through Thomas the Tank Engine and other toys, Yi Cui got used to hearing a refrain: "Daddy, I need you to change the batteries for me."
The boys have an unusually skilled helper in their father: Changing batteries has, in a sense, become a life's work for Cui, associate professor of materials science and engineering. In a busy laboratory at the Moore Building, he is pioneering the use of nanoscale materials like silicon and sulfur, rarely or never before incorporated into batteries. His group is building and testing batteries that last longer, store more energy and charge faster—all while keeping prices low.
During a recent visit, hundreds of experimental batteries, encased in stainless steel and resembling so many oversize coins, were slotted into machines to test their endurance as they constantly charge and discharge. (If the batteries last for months, it's a good sign.) Other machines evaluate how the batteries perform in different conditions, such as cold and heat. The idea, ultimately, is to create batteries that will allow mobile phones to last longer, electric cars to travel farther on a single charge, and the power grid to make use of renewable energy even when the sun isn't shining and winds aren't blowing.
"You start to ask how much more energy you can pack in for a given [battery] size or weight," Cui said at a Stanford energy seminar this year. "If we put in a lot more, would we be able to generate a new revolution in the technology, mainly in transportation?" Cui saw portable electronics as another area ripe for change: With better batteries, devices could offer more features, last longer and possibly take on different shapes, as people seek wearable devices with high computing power.
This is ambitious, but Cui, 38, is an astonishingly prolific researcher. (His group has produced at least 40 papers annually since 2011.) Since coming to Stanford in 2005, Cui has worked on a variety of projects, including solar cells and the use of nanoscale paper and textiles as a basis for making everything from ultracapacitors to water filtration technologies. But his battery work has made the biggest splash, starting in 2007 when he produced a widely cited paper on the use of silicon nanowires to increase batteries' energy storage capacity. Months later, he got a $10 million grant from Saudi Arabia's King Abdullah University of Science and Technology, a sum that "made everything fly much faster."
Last year, a Sunnyvale company Cui founded, Amprius, began shipping batteries in China that are used in the ThL cell phone sold there. "It has 20 percent more energy [for the same volume] than the best iPhone batteries on the market—the iPhone 5s," Cui says, as he holds one of the batteries in his palm. Plucked out of the back of a demonstration phone, the battery is about the size of a credit card, but thicker. Already several hundred thousand batteries have been sold, and the phones retail for about $300 each, according to Cui. "This is our generation one," he says of the battery. So far, it is his only product in commercial use.
Cui grew up in Guangxi province in southern China, where his mother taught Chinese at an elementary school and his father was a high school chemistry teacher. Around second or third grade, after school let out, he sometimes wandered into his father's classroom. Occasionally he got to see a chemistry experiment where "all these beautiful colors change," he recalls, a touch wistfully, from his office in the McCullough Building. That, he adds, is how he fell in love with the subject.
Cui studied chemistry at the University of Science and Technology of China (the country's equivalent of Caltech), and after graduating in 1998 he headed to Harvard for more. This was just after the dawn of nanotechnology, the idea that extremely tiny, atomic-level materials have shapes, colors, movements and behaviors that differ from those of larger materials. One example that fascinated Cui early on was gold. "You look at gold—it's gold in color," he explains. "When you make it into small-size particles, you can disperse them into solution. . . . It's a red color!" Nanomaterials, Cui reasoned, could become to our age what stone and ceramics were to other eras: breakthrough materials that could spur innovation.
During his Harvard PhD work and a subsequent postdoc at Berkeley, Cui studied under some of the greats: Charles Lieber, a Harvard chemistry professor who shaped the field of nanowires, a type of tiny building block; and Paul Alivisatos, a Lawrence Berkeley National Laboratory expert in nanocrystals, which are a different structure that can come in spherical or cubic shapes. Both men were among the first generation of scientists to study nanomaterials, after powerful machines commercialized in the late 1980s made it possible to move atoms around. This era, the late 1990s and early 2000s, had "a lot of new science coming out," Cui remembers. "Every couple of weeks, you see something jumping out [and think], 'This is amazing.'"
When Cui arrived at Stanford in 2005, he was determined to solve real-world problems using nanoscience. Years of hard work had taken their toll—after completing his PhD, he had gone through a brief burnout period—but at Stanford, he re-energized himself by approaching his research in a different way. Previously, he had explored the properties of nanomaterials and then figured out how those properties could be useful, a type of thinking he calls "left-to-right." But he was eager to change things around, first deciding what real-world issues needed to be addressed and then figuring out how nanomaterials could solve them.
The top three problems, he concluded, were energy, the environment and human health. Energy in particular was a growing concern in 2005, when oil and gas prices were starting to soar, making solar power and other forms of renewable energy more desirable. The Achilles' heel of renewables—the inability to provide backup power in the absence of sun or wind—was obvious. More people were also turning to hybrid-electric cars to avoid expensive gasoline, and demand for portable electronics was growing insatiably.
After poring over papers and books, Cui decided to tackle improving batteries. He says he didn't know much about them at the time.
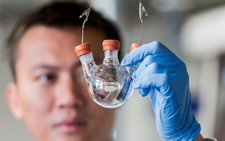
At its simplest, a battery involves the transfer of electrons between an anode, which wants to lose them, and a cathode, which likes to gain them. A substance called an electrolyte, situated between the anode and the cathode, conducts positive ions, which are atoms (or molecules) that have lost electrons. The ions move to the anode when the battery is charging, and to the cathode when discharging. From the anode, the electrons are forced into a wire or equivalent structure, and the subsequent electricity can be used to power a lightbulb or turn on a cell phone. The battery thus converts chemical energy to electric energy, and is a means of storing energy. Sometimes, Cui makes batteries at home with his older son, using an apple or other piece of fruit as the electrolyte. (The fruit's acid allows it to conduct ions easily.) The best batteries, he says, optimize a number of factors: safety, cost, temperature, energy, power (how quickly they charge and discharge), life cycle (number of charges) and longevity (how many years they last).
For more than a century, innovation was relatively stagnant as the lead-acid battery, invented around 1859 by a French scientist, dominated the field. Lead-acid batteries existed in the earliest automobiles, which were powered by electricity during the late 19th century, and they are still used to start engines in modern cars, though the heavy-duty work of propelling the vehicle now falls to energy-dense gasoline. Nickel-cadmium batteries, invented in the late 19th century, powered some of the first laptops.
But in the late 1980s and early 1990s, commercial electronics began to proliferate, driving the need for lighter, more energy-dense batteries than the existing chemistries provided. Lithium-ion batteries, now found in portable electronics and electric cars like the Tesla, began creeping onto the market around 1991. So did others, such as nickel metal hydride batteries, the type found in the Toyota Prius hybrid. Lithium is a good material for batteries because it is a lightweight solid (it is the third-lightest element in the periodic table), abundant in salty lakes and the ocean, and eager to lose its electrons to become an ion. Compared with lead-acid batteries, a lithium-ion battery can store five times as much energy per unit weight, says Cui.
Amid the flurry of work done in the past 10 or 20 years, commercial lithium-ion batteries have improved to the point where they have reached the limits of fundamental chemistry. Lithium is still desirable, but using graphite as an anode and cobalt-oxide as a cathode, as most commercial batteries do, will no longer deliver the steady energy gains of the past decade.
"To have a jump, you really need new materials," says Cui. For some battery structures, he noted at the energy seminar this year, there is now "a whole zoo of battery chemistry you can explore."
In the lab at Moore, Cui began trying to incorporate silicon into an anode. Found in sand, silicon is theoretically ubiquitous, but extracting nanoscale particles of it is expensive. Cui is looking at rice husks, which have compounds that become silicon oxide distributed throughout their biological cells, as a source. "You can just burn away the organics, and what's remaining is this silicon oxide nanoparticle," he explains. A silicon anode can store more lithium, hence considerably more energy, than batteries commonly used today.
To build cheaper anodes and cathodes, researchers in Cui's group have also been trying to extract tiny carbon fibers from materials as simple as crab shells. Once, some of them bought stone crabs at a Chinese grocery store in California. They burned them so that just the calcium-carbonate shell remained, then made a powder from it. After more tinkering, they emerged with hollow carbon nanofibers.
For most of the past decade, Cui and his large team of graduate students and postdocs (35 currently work at the Moore lab) have built different silicon structures to incorporate into batteries. The goal is to keep the silicon together: It's a brittle material, prone to stretching when it reacts with lithium and then breaking as the battery charges. It also has a bad habit of reacting with the electrolyte in the battery, which creates "gunk" and harms performance.
To prevent the silicon from breaking, Cui and his team tried different configurations, including silicon nanowires and silicon particles that were too small to break. They developed an "egg" structure that gave the silicon, encased loosely like a yolk in a carbon shell, some room to bulge without cracking the coating. In February, they announced the development of a "pomegranate" structure, in which the seed-like "eggs" are gathered up into clusters within a larger carbon "rind," thus reducing the contact between silicon particles and the electrolyte. "With the seventh generation of this design, we finally feel like silicon is getting there," Cui told the energy seminar audience. The next steps are to reduce the work required to make the pomegranate structures on a large scale, and find a way to cheaply extract silicon nanoparticles from rice husks or another source. (Amprius, Cui's company, is already using silicon anodes commercially.)
Batteries are a tough and expensive field to crack, and several high-profile companies have gone bankrupt in the last few years. But Cui is undaunted, and last year he began working with former U.S. Secretary of Energy Steven Chu on another battery, featuring a lithium-metal anode that Cui describes as the "holy grail" of energy storage technology because of its enormous capacity. Chu, a professor of physics and of molecular and cellular physiology, doesn't work in the lab but provides advice; he has, says Cui, "a lot of ideas about batteries."
Cui is also focusing on sulfur for a battery cathode. Sulfur is widely available and has a high capacity, so a lithium-sulfur combination could pack four to six times as much energy as existing battery technology. But Cui says sulfur is also "very challenging" because of conductivity problems. It can dissolve into the electrolyte. Lithium-sulfur batteries have been tried in unmanned vehicles but little else. Cui is pressing ahead: Having tried different shapes and forms to overcome the shortcomings of silicon, he wants to use the same strategy for sulfur.
With automakers and electronics companies clamoring for the next generation of lightweight lithium batteries, improvements may come quickly. Amprius, which recently added Chu to its board, has already commercialized its lithium-silicon battery. "It's likely in 10 years we can have the Tesla drive 500 [miles]"—about double its range now, says Cui, who still drives a 12-year-old car but says his next will probably be an EV. The trick will be keeping the battery cheap and lightweight.
Reshaping the electric grid represents another huge challenge, with enormous stakes. Renewable energy, especially solar power, is touted as a key part of the solution to climate change and energy independence. Solar growth has been rapid as prices for the Chinese-made panels have plunged. Always drawn to the cutting edge, Cui has put plenty of time into the solar problem, in addition to batteries. One second-year PhD student in his lab, Thomas Hymel, demonstrated a high-efficiency solar cell so thin and flexible it can be rolled "like a carpet," in Cui's description, allowing it to more easily adhere to a bumpy surface, among other advantages. "If you can convert just .01 percent of the energy coming to the earth every day," says Cui, "that can power the whole world."
But if solar power is going to get big, the ability to store energy for use in non-sunny periods will become of paramount concern. Right now, incredibly, the most common large-scale energy storage systems in the United States are hydroelectric plants. Water is pumped uphill during times of excess energy and then released through hydropower turbines when the electricity is needed. It's a cumbersome process, and it's not possible in places without dams. In the lab, Cui is testing semiliquid batteries—lithium metal in a polysulfide solution, in which the liquid reacts with the lithium during charging. Any applications for the grid need to be "very low-cost and highly scalable," he says.
Down the line, Cui has an even bigger vision for batteries, even the common ones that power phones and toys. "I think it would be really cool to do wireless charging in the future," he says dreamily. However, "I haven't worked on anything like that yet."
Kate Galbraith is a San Francisco-based energy and environment journalist and co-author of The Great Texas Wind Rush.
Batteries: An Inside Story
If Cui succeeds in making the new materials work, it will be partly because he can see inside the experimental batteries while they are operating. This "in situ" technology, providing extraordinary black-and-white images, has become readily available only in the past several years and is improving all the time. High-energy, extra-bright X-rays generated at the SLAC National Accelerator Laboratory can capture a material expanding and breaking, helping researchers probe batteries closely. The X-rays travel through helium-filled pipes so that they are not absorbed too soon, and then get focused through a condenser lens on the tiny battery sample that had been prepared in an argon-filled box, away from moisture and oxygen.
Johanna Nelson Weker, an associate staff scientist at the Stanford Synchrotron Lightsource within SLAC, has been x-raying batteries for Cui and others for more than three years. She has seen some fascinating things. One battery, made from the element germanium, expanded and contracted before her eyes, as the lithium ions moved between the cathode and anode while the battery charged and discharged. "We can watch them essentially breathe," she says. "As you lithiate them, they expand, and as you de-lithiate them, they contract." A recent X-ray image showed one of Cui's new experiments with silicon particles in a self-healing polymer—the silicon expands and breaks the polymer, which mends itself. The image was black-and-white and appeared to a lay viewer the way clouds do from a plane. Soon, Weker hopes to add another dimension to the images, so that they will appear in color, reflecting their chemical state.
With that ability, researchers could "see where the lithium is going, really," she says. "And that's what everybody wants to know. We can't actually see the lithium, but we can see this interact with the metal." The goal is to "see the lithium basically changing a particle from red to green as it lithiates, and then green to red as it de-lithiates."
The trick is "getting the information you want without damaging your sample," Weker says. That means making sure the batteries are not overexposed to radiation through too frequent X-rays. Some samples stay in the X-ray booth for more than a day, with X-rays running every half-hour.
Electron microscopy, available at Stanford rather than SLAC, produces even higher-resolution images of atoms. However, in order to absorb the electrons, the batteries must be so thin that they are "not close to a normal operating battery," Weker says. Still, she says, the technique is good for very thin materials such as the silicon nanowires Cui has used as a building block.
Cui is bullish on imaging technology. "It can further improve," he says, but "even with the current capability, there's a lot of battery problems we can study."
1. What's That You're Wearing? Nanotechnology makes paper and cloth batteries possible (2010)
3. SAGE: What is the best way to dispose of used alkaline batteries? (2011)